Experimental testbeds are proving that the principles of the quantum internet are functional, and some components are approaching maturity. But turnkey systems are another five to ten years away from performing.
ANDREAS THOSS, CONTRIBUTING EDITOR
The internet is an inescapable part of modern life, and the hardware that enables it is almost as ubiquitous. Fiber cables transmit terabytes of data around the world every second and, although the technology is constantly evolving, it has become almost commonplace. The growing interest and investment in quantum technology, however, promises to enable an evolutionary leap in internet technology. But will this quantum internet replace or build upon the current internet? Will it emerge anytime soon? And what might it look like?
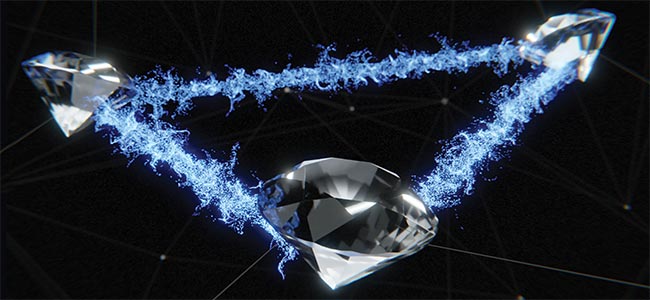
Artist’s impression of a three-node quantum network. The diamonds allude to one of the ways to generate the single photons on which the quantum internet relies — namely, the use of color centers in diamond crystals. Courtesy of Matteo Pompili, QuTec.
Regarding the first question, it would be easier for quantum internet developers to deploy as many ideas as possible from the old world to the new realm. On the hardware side, the quantum internet would still involve the transmission of photons via optical fibers, for example, though some changes would be necessary. Glass fiber can transmit quantum signals, but there are substantial differences between transmitting current internet signals and quantum communications. First, the quantum internet relies on the transmission of single photons that cannot be amplified or split. Without amplification, these signals diminish within 100 km or less of their source. Therefore, optical loss and noise present much bigger problems for the quantum world.
Further, the strange behavior of entanglement occurs, in which some aspects of one photon correlate to the same aspect of another, regardless of how distant they are from each other. One entangled photon, for example, might have a polarization state of A, while the polarization state of its entangled twin might be B, which is perpendicular to that of A. Until the state of a photon is measured, it can only be predicted based on certain probabilities. But measuring the state of one entangled photon discloses the state of the other. Einstein and his contemporaries were puzzled by this theoretical behavior in the 1930s. The experimental proof to support that the states of entangled photons remain undefined — or a mix of A and B — until they are measured emerged much later and eventually proved to be worthy of the Nobel Prize in physics in 2022.
These principles cause the quantum internet to be much more difficult to build than the current internet, but there are good reasons to make the attempt. A quantum internet could transmit data much more securely with the use of single photons. Or entangled photons could link quantum computers to be even more powerful. Quantum computers perform calculations by combining quantum states that are packaged as information into qubits. A fixed qubit in a quantum computer can be entangled with a photon to create a “flying qubit” that can be transmitted to another quantum computer in real time. There, flying qubits could control secure calculations that not even the operator of that computer could see.
Single-photon sources
If the future quantum internet relies on photons to exchange information, then three basic component technologies would be necessary to achieve this: a coherent light source, a system for transmitting photons, and a detector. A means for storing quantum information would also be helpful, but storing photons is still a big challenge.
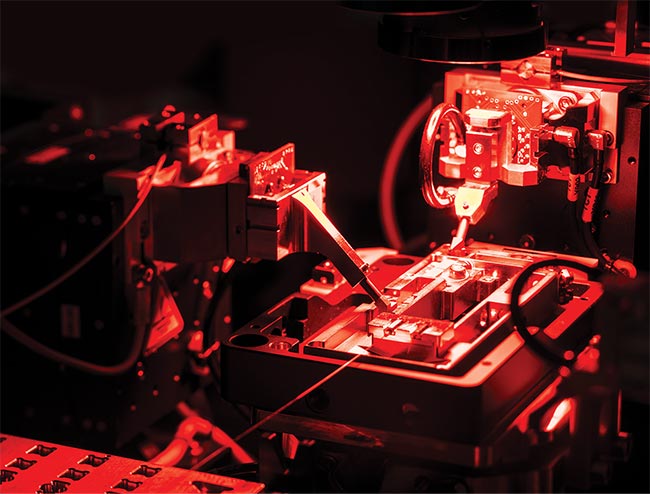
The future of quantum components will see increasing integration and automated manufacturing. Here, an entangled photon pair source is manufactured at Quantum Optics Jena. Courtesy of Quantum Optics Jena.
Detecting a single photon simply requires a low-noise detector, though detecting the quantum state of a single photon requires an analytic setup. Developments in this quarter would help advance the quantum internet, but the biggest challenges involve the emission of single photons and their transport over long distances.
There are three common ways to generate single photons1. The first is to generate a weak coherent state by simply suppressing a laser beam. Samsung’s Galaxy Quantum smartphone applies such a photon source to enable quantum security based on random number generation.
The second type of single photon source produces individual photons via the excitation and subsequent photon emission from single atoms or molecules. Alternatively, the photon could come from engineered point sources, such as quantum dots or the color centers in diamond crystals.
The third approach delivers photon pairs, one of which might carry data while the other acts as a “herald” for triggering reception. Such heralded photons can be produced through nonlinear optical effects such as spontaneous parametric down conversion. This effect is the reverse of the second-harmonic generation effect that converts laser beams to shorter wavelengths. In spontaneous parametric down conversion, a UV photon passes through a nonlinear crystal and is converted into two red photons that are emitted at different angles.
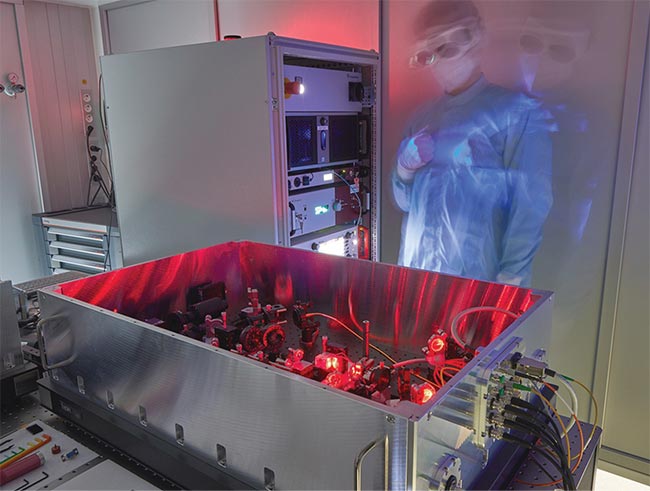
Performance testing of a quantum frequency converter at Fraunhofer Institute for Laser Technology (ILT). Such devices convert single photons from visible to infrared wavelengths to enable low-loss transport, or conversely, more efficient detection on quantum networks. Courtesy of Fraunhofer ILT.
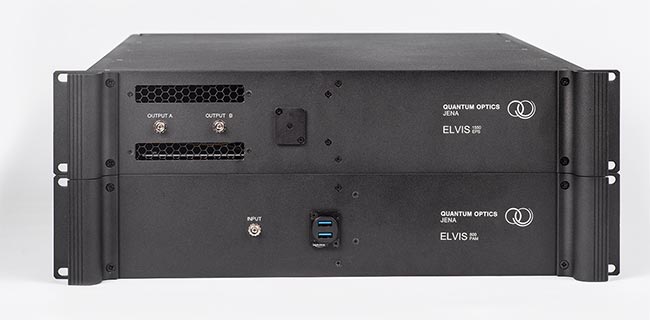
Certain elements of the quantum internet are already commercially available, such as quantum key distribution (QKD) solutions. Some rack-ready QKD systems incorporate entangled photon-pair sources and quantum-state analyzer systems. Courtesy of Quantum Optics Jena.
Single-photon sources can be evaluated by four criteria: purity (e.g., Does it emit only one photon, or more?); fidelity (e.g., How repeatedly do different photons adhere to an expected value?); efficiency (e.g., What percentage of photons can be used?); and the rate of photon generation. Different single-photon sources must meet different specifications. So, they cannot be evaluated by their parameters alone. They must match the requirements of the target application.
Still, it appears rather obvious that for quantum communication, the performance of single-photon sources should be as deterministic as possible.
Transport, convert, and repeat
The most important requirement for a single-photon transmission system is the minimization of optical loss. Any interface adds loss, and therefore individual single-mode fibers are the preferred transport solution. Free space transmission is also of interest, particularly for ad hoc or satellite connections.
The telecommunications industry has spent decades to refine low-loss optical
fibers. Thus, it is very sensible for quantum internet developers to try to use the infrared wavelengths commonly used for telecom bands. This is a challenge for most single-photon sources, such as nitrogen voids in diamonds (known as NV centers), because these sources emit in the visible spectrum.
Fortunately, there are nonlinear effects that allow the conversion of visible-wavelength photons to the common telecom band at 1.5 µm. To achieve this, the source photonic signal is sent to a nonlinear crystal along with a strong optical pump pulse. This efficiently converts the signal into the desired band based on either the sum or difference of each source’s frequency. A team of researchers from QuTech in the Netherlands and Germany’s Fraunhofer Institute for Laser Technology (Fraunhofer ILT) developed a quantum frequency converter that they reported had an external efficiency (fiber in/fiber out) of roughly 50% as well as ultralow noise of 2 Hz/pm.
Scientists at QuTech have also tested quantum repeaters, a component technology that qualifies as a holy grail for the quantum internet since it would allow quantum signals to be sent over long distances in fiber. In the current internet, repeaters measure an incoming
optical signal, copy it, and send out a much stronger version. The quantum internet, however, uses single photons, which conventional repeaters cannot amplify or measure. The measurement of photonic states — particularly entangled states — effectively collapses those states.
Quantum repeaters might overcome this challenge by generating entangled photons at two points, A and B, on either side of a third point, C. A and B send their entangled signals to C, where the signals are measured with respect to each other. The results of the measurements are sent back to A and B to understand how their respective photons relate to each other. This procedure is called entanglement swapping and can be repeated several times over long distances.
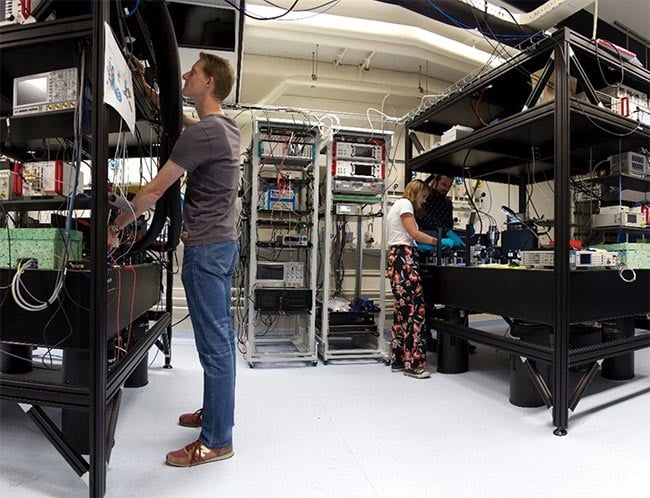
A two-node setup in the laboratory of QuTech in Delft, Netherlands. The team recently moved the second node to The Hague to test quantum protocols over a 30-km connection. Courtesy of QuTech.
Quantum repeaters also require a means to store quantum information, which is even more challenging. A photon and its state can be stored in atoms that the photon excites. If the stored photon is needed for a measurement, such as a comparison with another photon, then a readout signal is sent to induce the emission of a photon that has essential properties of the one that is stored.
Ronald Hanson, one of the founders of the Dutch QuTech institute, has tested quantum repeaters based on NV centers, which were not that effective. The system delivered entangled photons about every second. “We’re already working on next generation platforms that we believe can be made more efficient,” he said.
A layered quantum internet
Similar to today’s internet, the quantum internet could group system functions into layers, in which each layer solves specific tasks. Such services are provided to the layers above, reducing complexity for the higher layers.
Within the current internet, layers are defined in the Transmission Control Protocol/Internet Protocol (TCP/IP) stack or in the Open Systems Interconnection (OSI) model. In very simple terms, the quantum internet might incorporate an application layer on top of where internet users submit general tasks. Below that, within layer two, electronic computing translates the task into routines that can be executed by layer three, the physical (photonic) layer, in which photons are generated and measured2. Notably, as each layer’s complexity grows, more layers might need to be added.
Most network installations start with a simple task and add complexity along the network’s development path. The simplest level of functionality is seen in trusted node networks, in which only quantum keys (i.e., photon sequences) are exchanged. This process works even without entanglement.
The next level is sometimes referred to as the “prepare and measure network,” because it involves sources that prepare quantum states and devices to measure them. Advanced communication protocols can be tested there. A fully functional entanglement distribution network assumes the generation of entangled photons in a deterministic way. Adding quantum memory to this stage would enable the implementation of much more complex protocols and, in fact, provide entry to
the world of quantum computing. For
efficient quantum computing, the networks that link computers must deploy a means for fault-tolerant operation.
Systems and installations
There are many projects on the regional, national, and international level that are helping to evolve quantum communication technology. A comprehensive overview of each of them would require a separate article, but most share common strategies that focus on improving functionality, transmission distance, and scaling the number of network nodes.
After a new functionality is tested in the lab, it is field-tested by setting up a distant node connected to the original lab via dark fibers — installed but unused telecommunication fibers.
At QuTech, for example, researchers connected two quantum nodes in the lab and exchanged entangled photons before scaling up the number of nodes.
“We have three nodes connected, and we can do quantum information protocols on this little network,” Hanson said. According to Hanson, the team later extended its network’s reach. “We have one of these quantum processors here in Delft, and one was moved to The Hague just last month [in May]. They are connected over 30 km of deployed fiber. We plan to test this summer that we can actually generate entanglement between these processors, but now through 30 km of fiber in the ground.”
Similar efforts are underway in the U.S., Canada, China, and Europe. Germany’s QuNET initiative is one of the largest research projects working on secure quantum communication. The plan is to establish secure communication links between governmental institutions across Europe. Similarly, Fraunhofer’s Heinrich Hertz Institute (HHI) is employing a quantum communication testbed to explore the potential of quantum communications with today’s internet infrastructure. In one experiment, HHI developed a testbed infrastructure to connect quantum communication with 5G and 6G networks, according to Nino Walenta, a project team leader at HHI. “This helps people to test applications on quantum encrypted next-generation networks,” he said.
Although quantum technologies are developing rapidly, standardization
efforts to ensure their compatibility are still evolving. Standards for quantum encryption are already quite advanced, and government metrology institutes are working hard to establish standards for single-photon measurements. But interestingly, the global community often still relies on different terms and definitions to express similar concepts. This problem was recognized, and last year a group of researchers at the National Institute of Standards and Technology (NIST) in the U.S. began to establish a dictionary for single-photon source and detector technology.
“We are soliciting some input from the peers right now,” said Sergey Polyakov, a physicist at NIST and one of the authors of the dictionary, which is due for publication later this year.
Quantum internet technology research is admittedly scattered, and the efforts for its development are also very diverse at the moment, noted Mark Saffman, chief scientist for Quantum Information at Infleqtion Inc.
“The field is in a very developmental stage, and we’re not at the stage of having standardized designs with interchangeable components from different manufacturers,” he said. “I think every different place around the world where people have demonstrated these nascent quantum networks are using different designs, different components, different physical approaches. It’s all very much fluid at this time.”
References
1. C. Couteau et al. (2023). Applications of single photons to quantum communication and computing. Nat Rev Phys, Vol. 5,
pp. 326-338.
2. M. Pompili et al. (2022). Experimental
demonstration of entanglement delivery
using a quantum network stack. Npj
Quantum Inf, Vol. 8, p. 121.